An Unexpectedly Heavy and Complex Snowfall Event across the Southern Appalachian Region
by
David M. Gaffin, Stephen S. Parker, and Paul D. Kirkwood
ABSTRACT
- On 26 March 1999, an unexpectedly heavy and complex snowfall event occurred across the southern Appalachian region. This event produced 20 to 30 cm (8 to 12 inches) of snow across the Smoky Mountains and 10 to 15 cm (4 to 6 inches) across other portions of southwest North Carolina, northeast Tennessee, and southwest Virginia. This snowfall event was complex in that several different lifting mechanisms combined to produce unexpectedly heavy amounts, especially in a narrow band across the Great Tennessee Valley. Lift from frontogenesis, orography, cold air damming, and mesoscale waves contributed to the snowfall amounts across the entire region.
- An interesting aspect of this snowfall was the banded enhancements observed during the initial stage of the event. These banded enhancements, observed by both satellite and radar, were determined to be the result of mesoscale waves. These waves developed around 0900 UTC in the lee of the Smoky Mountains as a strengthening southerly flow above 850 hPa became nearly perpendicular to the Smokies. A moist stable layer just above the mountain ridges (between 850 and 650 hPa) provided a sufficient duct for mountain waves to form across northeast Tennessee. Convective activity later developed around 1200 UTC across northeast Georgia along an inverted surface trough. This convective activity appeared to have helped trigger additional waves across western North Carolina. It appeared that the waves contributed to the heavy snowfall amounts by providing additional lift to the larger scale lift present, which together maximized the release of the conditional instability across the region. After 1400 UTC, wave activity appeared to diminish across the southern Appalachian region as the larger scale lift overwhelmed the waves.
1. Introduction
- On 26 March 1999, a complex snowfall event occurred across the southern Appalachian region, producing unexpectedly heavy snowfall amounts (defined here as 10 cm (4 in) or more of snow within 12 h). The heavy snowfall totaled 20 to 30 cm (8 to 12 inches) across the Smoky Mountains and 10 to 15 cm (4 to 6 inches) across other portions of southwest North Carolina, northeast Tennessee and southwest Virginia (Fig. 1). Across the Great Tennessee Valley, downwind of the Smoky Mountains (Fig. 2), the heavy snowfall occurred in a narrow north-to-south band. During the initial part of the event (between 0900 and 1400 UTC), satellite and radar images revealed an interesting aspect to this event in that the precipitation was organized in west-to-east oriented bands which propagated to the north.
- In general, the development of banded precipitation can be attributed to several different processes, such as slantwise convection, frontogenesis, and mesoscale waves. Slantwise convection has been theorized to be the result of the release of conditional symmetric instability (CSI) in which an air parcel follows an unstable slantwise path in a conditionally and statically stable atmosphere (Emanuel 1983). Recent research has discussed the misuse of CSI in analyzing events with banded precipitation. Schultz and Schumacher (1999) highlighted the need to carefully consider any role that CSI may have played in an event, while also looking for other alternatives that better explain the data. Frontogenetical forcing has been known to create banding due to the development of secondary circulations in an environment in which CSI was present, and also in a weakly symmetrically-stable environment.
- The proximity of the Smoky Mountains to this area of snowfall indicated that mountain waves were also a possible cause of the observed banded precipitation. The southern Appalachian region contains terrain that is generally oriented from southwest to northeast and rises around 1500 m (5000 ft) above the surrounding valleys (Fig. 2). The Smoky Mountains contain some of the highest peaks in the southern Appalachians, around 1950 m MSL (6500 ft), with the Great Tennessee Valley stretching along the northwest side of the Smoky Mountains. Mountain waves are occasionally observed near the Appalachian Mountains and have been documented in previous research (Smith 1976). Queney et al. (1960) described several conditions necessary for mountain waves, including a cross-barrier wind flow that is oriented within roughly 30 degrees of a line perpendicular to the ridge line, cross-barrier wind speed exceeding a terrain-dependant value of 7 to 15 m s-1 (14 to 30 kt), and an inversion extending above the mountain ridge with weaker stability at higher levels in the upstream environment. Other factors which have been found to enhance the occurrence of mountain waves include wind speeds increasing with height (Scorer 1949; Colson 1954) and the existence of a mountain barrier with a gently rising windward slope and a steep leeward slope (Queney et al. 1960; Lilly and Klemp 1979).
- The snowfall event on 26 March 1999 was complex in that several lifting mechanisms combined to produce unexpected heavy amounts of snowfall across the southern Appalachian region. The purpose of this paper is to (1) determine the cause of the banded structure of the initial precipitation, (2) examine the lifting mechanisms that contributed to the heavier than expected snowfall, and (3) assess the ways operational forecasters can better anticipate this type of event.
2. Methodology
- For this study, the National Weather Service operational Eta model (Rogers et al. 1996), with a horizontal grid resolution of 32 km and 45 vertical levels on 26 March 1999, was primarily evaluated. The Eta model was chosen because it had the finest grid resolution of any operational model during this event, and its vertical coordinate system (step-mountain) was more similar to the actual terrain. The Eta (step-mountain) vertical coordinate system has been found to create less noise around mountainous terrain than the sigma (terrain following) vertical coordinate system (Mesinger et al. 1988). Other operational models, which use the sigma (terrain following) vertical coordinate system, were compared to the Eta, including the Nested Grid Model (NGM) (DiMego et al. 1992), the aviation model (AVN) (Parrish and Derber 1992), and the Rapid Update Cycle model (RUC) (Bleck and Benjamin 1993). On 26 March 1999, the NGM model had a horizontal grid resolution of 80 km with 16 vertical levels, the AVN model a horizontal grid resolution of 105 km (T126) with 28 vertical levels, and the RUC model a horizontal grid resolution of 40 km with 40 vertical levels. All of the model data were displayed using the General Meteorology Package (GEMPAK) software (desJardins et al. 1991) which interpolated the data for display with a grid resolution of 80 km.
- In order to assess whether the local environment was conducive for the formation of waves, several different methods from previous research were used. Koch and O'Handley (1997) introduced a duct factor analysis which helped determine the optimum area for wave formation due to an efficient ducting environment. This duct factor analysis was conducted using the following equation with model data:
DF =
(800) -
(950) +
e (800) -
e (400)
(1)
where
is the potential temperature and
e is the equivalent potential temperature at the pressure levels in parentheses. This duct factor analysis includes a measure of the strength of a conditionally unstable layer above a stable layer, which Lindzen and Tung (1976) found was necessary for the ducting of wave energy. While this analysis does not indicate whether the ducting layer is of sufficient depth, it does give the forecaster an idea of the optimum location where waves could form by showing the area with the best ducting environment (indicated by the greater duct factor values).
- Lindzen and Tung (1976) theorized that in order for waves to be maintained, a stable ducting layer must be adjacent to the surface and capped by a conditionally unstable layer. This conditionally unstable layer acts as a reflecting surface for the maintenance of waves when the mean flow equals the phase speed of the wave. Lindzen and Tung theorized that the stable ducting layer must be of sufficient depth according to the equation:
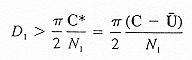
(2)
where D is the depth of the stable layer, C* is the mean flow-relative velocity, C is the observed phase velocity of the wave, U is the observed mean wind of the stable layer in the direction of wave propagation, and N is the Brunt-Vaisala frequency defined by:
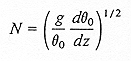
(3)
where g is the acceleration due to gravity and
is the mean potential temperature of the stable layer.
- Other wave research by Scorer (1949) theorized that mountain waves were a function of atmospheric stability and vertical wind shear according to the equation:
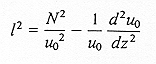
(4)
where l2 is the Scorer parameter, uo is the wind speed normal to the mountain ridge, and N is the Brunt-Vaisala frequency. Scorer theorized that if this parameter (l2) decreased with height, then mountain waves would be possible. Scorer also showed that in a two-layer atmosphere where l2 was constant within each of the layers, the necessary condition for the existence of mountain waves is:
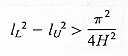
(5)
where lU and lL are the Scorer parameters in the upper and lower layers, and H is the depth of the lower layer.
3. Results
- i. Synoptic setting
- On the morning of 26 March 1999, a strong 500 hPa cut-off low was slowly moving southeast across the Ohio and Tennessee Valleys (Fig. 3). This upper low created southerly flow above the Smoky Mountains (above 1800 m elevation) with northeast flow observed in the boundary layer, as evidenced by the Velocity Azimuth Display (VAD) wind profile (Fig. 4) from the Morristown WSR-88D radar (KMRX) located in the Great Tennessee Valley. The Eta model predicted ascent across most of southwest North Carolina and northeast Tennessee at 1200 UTC (Fig. 5). Large height falls were also observed on the 300 hPa analysis (Fig. 6) at 1200 UTC. These results indicated that strong lift was occurring across southwest North Carolina and northeast Tennessee during the morning of 26 March 1999.
- Satellite images around 0915 UTC (Fig. 7a) revealed cloud-top temperature minima across northeast Tennessee as lift increased and light precipitation began. (satellite loop: 3.7 MB) Surface temperatures at the onset of precipitation (around 0915 UTC) were between 5 and 10 0C across northeast Tennessee and western North Carolina, suggesting the precipitation began as rain in the valleys precipitation began in an area devoid of surface observations). With dewpoint temperatures a few degrees below 00C, this initial precipitation likely created evaporative cooling across the area. In addition, adiabatic cooling likely resulted from the strong ascent in the stable atmosphere, ultimately changing the rain to snow. Across northeast Tennessee, banded areas of light precipitation, oriented west-to-east and propagating to the north, became evident around 0915 UTC on WSR-88D radar images from Morristown (KMRX) (Fig. 8a) and Greenville-Spartanburg (KGSP) (Fig. 9a). These banded areas of light precipitation on radar coincided with the banded enhancements observed on satellite images at this time. It should be noted that both KMRX and KGSP radars were in clear air mode (Volume Coverage Pattern (VCP) 31) around 0915 UTC, which increased their sensitivity to lower reflectivities. Also, some of the radar images appeared to indicate other banded echoes in a northwest-southeast or southwest-northeast orientation, but these apparent banded echoes were the result of beam blockage by the mountainous terrain. This beam blockage also obscured a few bands of precipitation in some of the radar images.
- By 1100 UTC, banded enhancements propagating to the north were evident on satellite images across northeast Tennessee (Fig. 7b). Radar images at this time (Figs. 8b and 9b) also continued to indicate banded areas of precipitation across northeast Tennessee and extreme western North Carolina. By 1100 UTC, both KMRX and KGSP radars were in precipitation mode (VCP 21), which reduced the sensitivity of these radars and changed the color scale. By 1246 UTC, satellite images (Fig. 7c) revealed additional banded enhancements across western North Carolina. Radars also continued to show some evidence of banded precipitation around 1245 UTC (Figs. 8c and 9c), although not as clearly as on the satellite images.
- The surface analysis at 1200 UTC (Fig. 10) revealed an inverted trough along the Georgia-South Carolina border extending into southern West Virginia, with evidence of cold air damming across the Carolinas. This surface trough became the focal point for the development of convective activity across northeast Georgia as lift increased across this area. Lightning strikes were first detected across northeast Georgia around 1152 UTC and continued until around 1552 UTC. This convective activity developed near the source region of, and at the same time as, the additional band enhancements which appeared across western North Carolina on satellite images around 1245 UTC. By 1415 UTC, satellite (Fig. 7d) and radar images (Figs. 8d and 9d) continued to show a large area of precipitation across the region, but no longer revealed evidence of banding.
- ii. Conditional symmetric instability and frontogenesis
- The banded appearance of the precipitation prompted an exploration into the potential for slantwise convection due to the release of conditional symmetric instability (CSI). Examination of vertical cross section data from the Eta model (Fig. 11) indicated that CSI was present, but that moist gravitational conditional instability (CI) was also present (evident by the layer of saturated equivalent potential temperature decreasing with height between 750 and 650 hPa). It is unlikely that slantwise convection occurred under these conditions, because the release of CI would dominate the release of CSI, as indicated by Bennetts and Sharp (1982). Also, if slantwise convection was occurring, the precipitation bands would likely be oriented along or within 15 degrees of the thermal wind (Schultz and Schumacher 1999). In this case, the bands were oriented nearly perpendicular to the thermal wind (inferred from the 850-400 hPa thickness field (Fig. 11a)). Therefore, slantwise convection due to the release of CSI was not the cause of the observed banded precipitation on 26 March 1999.
- Banded precipitation has also been associated with circulations generated by frontogenetical forcing. Examining the Eta model data at 1200 UTC on 26 March 1999, an axis of frontogenesis was occurring across the southern Appalachian region at the 800-hPa, 750-hPa, and 700-hPa levels (Fig. 12). This axis of mid-level frontogenesis was coincident with the inverted surface trough and the eventual observed area of heavy snowfall. The bands of precipitation produced by this frontogenesis would have been oriented parallel to the isotherms within the frontal zone (generally along a north-to-south axis). Thus, the observed bands of precipitation, oriented perpendicular (along an east-to-west axis) to the axis of frontogenesis and propagating to the north, were therefore not directly generated by frontogenetical processes. However, it is reasonable to assume that mesoscale lift from a transverse circulation associated with frontogenetical forcing led to an increase of instability in the region. This increased instability, in turn, could be released by the east-to-west oriented bands of precipitation as they propagated north across the region, resulting in heavier than expected snowfall in a relatively narrow north-to-south corridor. Therefore, frontogenesis most likely played an important role in this event, but was not the direct cause of the east-to-west oriented bands of precipitation early in the event (before 1400 UTC).
- iii. Waves
- The possibility of waves, observed as banded enhancements downwind of the Smoky Mountains around 1100 UTC (Fig. 7b) and later across the mountains of western North Carolina around 1245 UTC (Fig. 7c), was investigated using the several methods from the methodology section. First, the duct factor (Eq. 1) was calculated using model data to find the locations of the more favorable environments for the ducting of waves. It was determined, after examining the Eta model soundings over the southern Appalachian region at 1200 UTC, that the best pressure levels to define the stable layer for this event were the 700 and 850 hPa levels. The 700 and 400 hPa levels best defined the conditionally unstable layer. The resulting duct factor analysis (Fig. 13) revealed that the more favorable environment for the ducting of waves included most of northwest South Carolina, western North Carolina, northeast Tennessee, and southwest Virginia (DF>0 indicated a more favorable ducting environment). Although the optimum location for the ducting of waves was located east of the southern Appalachian region along the Atlantic coast, banded enhancements were not observed on satellite images in these areas. This was likely due to a lack of sufficient wave triggering mechanisms east of the southern Appalachian Mountains.
- The duct factor analysis also indicated that the 1200 UTC Greensboro, North Carolina sounding (Fig. 14) was generally representative of the environment across western North Carolina and northeast Tennessee. This can be inferred by the nearly identical duct factor values across both of these areas. The combination of the strength of the conditionally unstable layer and stable layer at the Greensboro sounding site was nearly the same as that observed across western North Carolina and northeast Tennessee. The 1200 UTC Greensboro sounding revealed that a moist, stable layer extended generally between 850 and 650 hPa, and was capped by a dry, conditionally unstable layer between 650 and 300 hPa. Another dry, conditionally unstable layer was situated adjacent to the surface below 850 hPa. This surface layer, combined with the mountainous terrain, could act as the lower reflecting surface for waves across western North Carolina. The dry, conditionally unstable layer above 650 hPa likely acted as the upper reflecting surface for the propagation and maintenance of waves in the stable layer between 850 and 650 hPa. This sounding profile was similar to the one that Lindzen and Tung (1976) found necessary for the ducting of wave energy.
- The Greensboro sounding was then evaluated to determine if the stable layer between 850 and 650 hPa was of theoretically sufficient depth to support waves. The potential temperature at the top of the stable layer was found to be 300 K, with the potential temperature at the bottom of the stable layer 280 K, yielding a Brunt-Vaisala frequency (Eq. 3) of 0.015 s-1. Using satellite imagery, the mean phase speed of the waves was estimated at 10.4 m s-1 (21 kt), which is similar to the mean wind speed of the stable layer in the direction of wave propagation of 7.0 m s-1 (14 kt) calculated from the KMRX VAD wind profile. The propagation of the waves to the north was found to coincide with the mean wind direction within the stable layer. Using Eq. 2, these parameters yielded a calculated ducting depth of 356 m, which is smaller than the observed stable layer depth of 2919 m. Thus, a stable ducting layer of theoretically sufficient depth in order to maintain waves was present the morning of 26 March 1999 across western North Carolina and northeast Tennessee.
- In order for mountain waves to occur, the observed wind flow in the stable layer above the mountain ridge must be nearly perpendicular (within 30 degrees) to the ridge. The highest mountain ridges in the southern Appalachian chain generally follow along a southwest-to-northeast axis. However, most of the mountain wave activity seen on the satellite images on 26 March 1999 appeared to originate around the highest ridges (above 1500 m) of the Smoky Mountains, which are oriented more west-to-east. Thus, the southerly flow observed (Fig. 4) above the mountain ridge tops was nearly normal (within 30 degrees) to the section of the Smoky Mountains that was oriented west-to-east, indicating that mountain waves were possible.
- The Scorer parameter (Eq. 4) was calculated and plotted every 100 hPa (Fig. 15) using the observed wind flow below 5.49 km from the 1200 UTC KMRX VAD wind profile. The observed wind flow above 5.49 km and thermal structure from the 1200 UTC Greensboro sounding were also used in the Scorer parameter calculations. With a south wind defined as being normal to the highest Smoky Mountain ridges, the calculated vertical profile revealed that the Scorer parameter decreased with height between 900 and 400 hPa. This result indicated that the conditions needed for mountain waves were present on 26 March 1999. The Scorer parameter was also recalculated assuming the observed south wind was as much as 30 degrees to the left of normal to the highest ridges of the Smoky Mountains. The fact that the vertical profile again decreased with height between 800 and 500 hPa (the layer immediately above the highest ridges of the Smoky Mountains) revealed that mountain waves were still possible.
- The possibility of mountain waves on 26 March 1999 was further examined using Scorer's theory of a two layer atmosphere. Using Eq. 5 and Fig. 15 and assuming that a south wind was normal to the highest Smoky Mountain ridges, lU (700 to 300 hPa layer) averaged 10 x10-7 m-2, lL (900 to 800 hPa layer) averaged 285 x10-7 m-2, and H was around 1800 m. Thus, the difference between the two layers (275 x10-7 m-2) was found to be greater than the lower layer depth (7.6 x10-7 m-2). This confirms that the necessary conditions for mountain waves were present the morning of 26 March 1999. Assuming the south wind was as much as 300 to the left of normal from the mountain ridges, lU (700 to 500 hPa layer) averaged 17 x10-7 m-2, lL (900 to 800 hPa layer) averaged 265 x10-7 m-2, and H was around 1800 m. The difference between the two layers (248 x10-7 m-2) was also found to be greater than the lower layer depth (7.6 x10-7 m-2). This further supports the idea that as the southerly wind flow strengthened with the approach of the strong upper-level low, the initial banded precipitation observed around 1100 UTC (Fig. 7b) was the result of mountain waves trapped in the moist stable layer between 850 and 650 hPa across northeast Tennessee.
4. Discussion
- Because of the mesoscale nature and short duration of these mountain waves, most operational hydrostatic models, with grid resolution of 32 km or greater, cannot resolve them. The quantitative precipitation forecast (QPF) by the 0000 UTC initialized short-range models (Fig. 16) underestimated the liquid-equivalent amounts observed between 1200 and 1800 UTC (assuming a snow/liquid ratio of 10:1). The model that was most accurate compared to the observations was the Eta model, which accurately predicted the location of the heavy snowfall across the Great Tennessee Valley. This was likely due to its better forecast of the frontogenetical circulation. Despite its forecast over the Great Tennessee Valley, the Eta's 6-hour QPF totaled only 0.38 cm (0.15 inches) of liquid-equivalent precipitation, or around 3.8 cm (1.50 inches) of snow between 1200 and 1800 UTC (when most of the observed snow fell). Orographic lift likely accounted for a significant amount of the snowfall observed along the mountain ridges, estimated to be around 10 to 20 cm in the Smoky Mountains (Fig. 1) and around 5 to 15 cm of the snowfall amounts observed along the Virginia-Kentucky border. Another lifting mechanism responsible for a large percentage of the snowfall across western North Carolina east of the inverted surface trough, and some of the snowfall across the Great Tennessee Valley along the inverted trough, was isentropic lift due to cold air damming. Cold air damming has been well documented across the Carolinas (e.g. Forbes et al. 1987). The inverted trough across northeast Tennessee was likely the western edge of the cold air damming on 26 March 1999.
- The snowfall amounts in excess of 10 cm, reported in a narrow band across the Great Tennessee Valley near Morristown (mainly during the 1200 to 1800 UTC time period), were primarily the result of lift produced from a combination of mountain waves, frontogenesis, and cold air damming. In addition, the wave activity observed across western North Carolina after 1200 UTC may have aided in the snowfall amounts over this area. The ascent region of the mountain waves provided additional lift to the larger scale lift present, which was superimposed on the mesoscale ascent/descent regions generated by the waves. These waves may have maximized the release of the conditional instability present across the region, which could have aided in the heavier observed snowfall amounts compared to the Eta model's QPF. Thus, it appeared that the wave activity enhanced the observed snowfall across the Great Tennessee Valley and a part of western North Carolina, although surface observations were not located underneath the path of these waves in order to verify. Bosart and Sanders (1986) also found difficulty in proving precipitation enhancement by waves, but concluded that strong fluctuations in the column total condensation rate occurred with the passage of a gravity wave in a major Atlantic Coast snowstorm.
- Operational forecasters can identify the potential for wave activity by examining local soundings for the presence of a stable layer extending above the mountain ridges and situated underneath a conditionally unstable layer. An automated duct factor analysis could alert operational forecasters to the optimum area for development of waves. After observing a moist stable layer extending above the highest mountain ridges, mountain waves could be expected if the cross-barrier wind flow was expected to become within 30 degrees of normal to the mountain ridges, exceed 7 to 15 m s-1 (14 to 30 kt), and increase with height. Also, the fact that the Smoky Mountains exhibited a gentle rising windward slope and a steep leeward slope with a southerly wind enhanced the probability of mountain waves. Once mountain waves have been anticipated, procedures using the Scorer equations and local soundings on the Advanced Weather Integrated Processing System (AWIPS) (National Weather Service 2002) could be activated to further determine the likelihood of waves.
- Furthermore, the potential for development of other wave-generating mechanisms such as thunderstorms, geostrophic adjustment processes, and shear instability (Uccellini and Koch 1987) should be analyzed to determine if the possibility for wave activity could be further enhanced across the forecast area. By anticipating the development of favorable wave-ducting environments and wave-generating mechanisms, operational forecasters can at least recognize the potential for wave events such as that observed on 26 March 1999. This better recognition would be beneficial in subsequent briefings and weather statements, resulting in quicker and more accurate adjustments of the forecast if waves were observed.
5. Summary
- Several lifting mechanisms combined on the morning of 26 March 1999 to result in a complex snowfall event with heavier than expected amounts. The interaction of the different lifting mechanisms created observed snowfall that was greater than twice the amount of the short-range model's QPF. Frontogenetical forcing and CSI were found to be present across the Great Tennessee Valley, and played an important role in the production of the heavy snowfall. In addition, orographic lift along with isentropic lift due to cold air damming appeared to have contributed to a large portion of the snowfall amounts across western North Carolina and some of the snowfall amounts across northeast Tennessee. The most interesting aspect of this event was the banded enhancements observed on satellite and radar images during the initial stage of the event. These banded enhancements were determined to be the result of waves. The initial mountain waves observed across the Great Tennessee Valley appeared to have contributed to the heavy snowfall amounts by providing additional lift to the larger scale lift in place. This larger scale lift was superimposed on the mesoscale ascent/descent regions generated by the waves. The increased lift in the ascent region of the waves may have also maximized the release of the conditional instability observed across the region, ultimately resulting in heavier than expected snowfall amounts across the Great Tennessee Valley. Additional wave activity formed across western North Carolina after 1200 UTC, which appeared to be the result of convective activity over northeast Georgia. This wave activity may have also enhanced the snowfall amounts across western North Carolina.
- By recognizing the necessary conditions for the formation of waves, forecasters may be able to better anticipate and identify them. In this case, an examination of the morning soundings indicated that a moist stable layer extended above the highest ridges of the Smoky Mountains, a southerly wind was nearly perpendicular to these ridges, and wind speeds increased with height. Also, the Smoky Mountains provided a gentle rising windward slope and a steep leeward slope with this southerly wind, which enhanced the possibility of mountain waves. New technology, such as AWIPS, may allow operational forecasters to develop procedures to quickly calculate a duct factor analysis in order to locate the optimum area for the development of waves. Additional procedures could also help operational forecasters further evaluate the potential for waves by calculating the Scorer and Lindzen and Tung equations from local soundings.
REFERENCES
- Bennetts, D. A., and J. C. Sharp, 1982: The relevance of conditional symmetric instability to the prediction of mesoscale frontal rainbands. Quart. J. Roy. Meteor. Soc., 108, 595-602.
- Bleck, R., and S. G. Benjamin, 1993: Regional weather prediction with a model combining terrain-following and isentropic coordinates. Part I: model description. Mon. Wea. Rev., 121, 1770-1785.
- Bosart, L. F., and F. Sanders, 1986: Mesoscale structure in the megalopolitan snowstorm of 11-12 February 1983. Part III: a large-amplitude gravity wave. J. Atmos. Sci., 43, 924-939.
- Colson, D., 1954: Meteorological problems in forecasting mountain waves. Bull. Amer. Meteor. Soc., 35, 363-371.
- desJardins, M. L., K. F. Brill, and S. S. Schotz, 1991: GEMPAK 5 Part I-GEMPAK 5 programmer's guide. National Aeronautics and Space Administration. [Available from Scientific and Technical Information Division, Goddard Space Flight Center, Greenbelt, MD 20771.]
- DiMego, G. J., K. E. Mitchell, R. A. Peterson, J. E. Hoke, J. P. Gerrity, J. J. Tuccillo, R. L. Wobus, and H. -M. H. Juang, 1992: Changes to NMC's Regional Analysis and Forecast System. Wea. Forecasting, 7, 185-198.
- Emanuel, K. A., 1983: On assessing local conditional symmetric instability from atmospheric soundings. Mon. Wea. Rev., 111, 2016-2033.
- Forbes, G. S., R. A. Anthes, and D. W. Thomson, 1987: Synoptic and mesoscale aspects of an Appalachian ice storm associated with cold-air damming. Mon. Wea. Rev., 115, 564-591.
- Koch, S. E. and C. O'Handley, 1997: Operational forecasting and detection of mesoscale gravity waves. Wea. Forecasting, 12, 253-281.
- Lilly, D. K., and J. B. Klemp, 1979: The effects of terrain shape on nonlinear hydrostatic mountain waves. J. Fluid Mech., 95, 241-261.
- Lindzen, R. S. and K. K. Tung, 1976: Banded convective activity and ducted gravity waves. Mon. Wea. Rev., 104, 1602-1617.
- Mesinger, F., Z. I. Janjic, S. Nickovic, D. Gavrilov, and D. G. Deaven, 1988: The step-mountain coordinate: Model description and performance for cases of alpine lee cyclogenesis and for a case of an Appalachian redevelopment. Mon. Wea. Rev., 116, 1493-1518.
- National Weather Service, 2002: AWIPS information. [Available online at https://www.awips.noaa.gov/].
- Parrish, D. F., and J. C. Derber, 1992: The National Meteorological Center's spectral statistical-interpolation system. Mon. Wea. Rev., 120, 1747-1763.
- Queney, P., G. Corby, N. Gerbier, H. Koschmieder, and J. Zierep, 1960: The airflow over mountains. WMO Tech. Note 34, 135 pp. [Available from World Meteorological Organization, P.O. Box 2300, CH-1211 Geneva 2, Switzerland.]
- Rogers, E., T. L. Black, D. G. Deaven, G. J. DiMego, Q. Zhao, M. Baldwin, N. W. Junker, and Y. Lin, 1996: Changes to the operational "early" Eta analysis/forecast system at the National Centers for Environmental Prediction. Wea. Forecasting, 11, 391-413.
- Schultz, D. M. and P. N. Schumacher, 1999: The use and misuse of conditional symmetric instability. Mon. Wea. Rev., 127, 2709-2732.
- Scorer, R., 1949: Theory of waves in the lee of mountains. Quart. J. Roy. Meteor. Soc., 75, 41-56.
- Smith, R. B., 1976: The generation of lee waves by the Blue Ridge. J. Atmos. Sci., 33, 507-519.
- Uccellini, L. W. and S. E. Koch, 1987: The synoptic setting and possible energy sources for mesoscale wave disturbances. Mon. Wea. Rev., 115, 721-729.
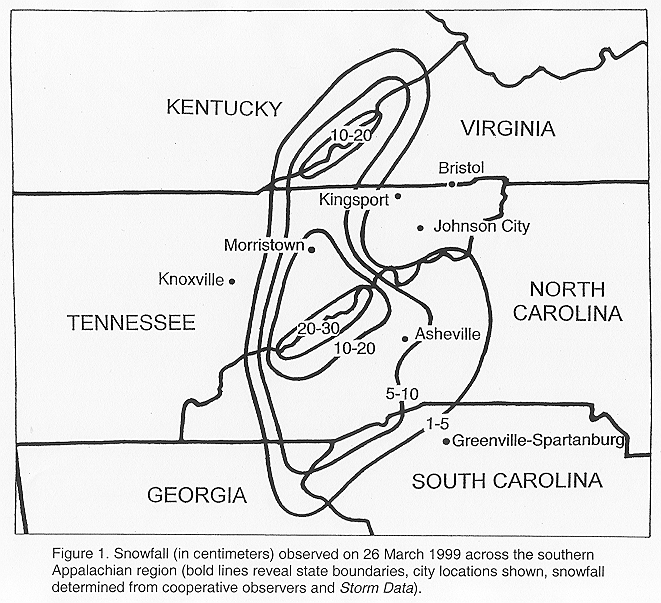
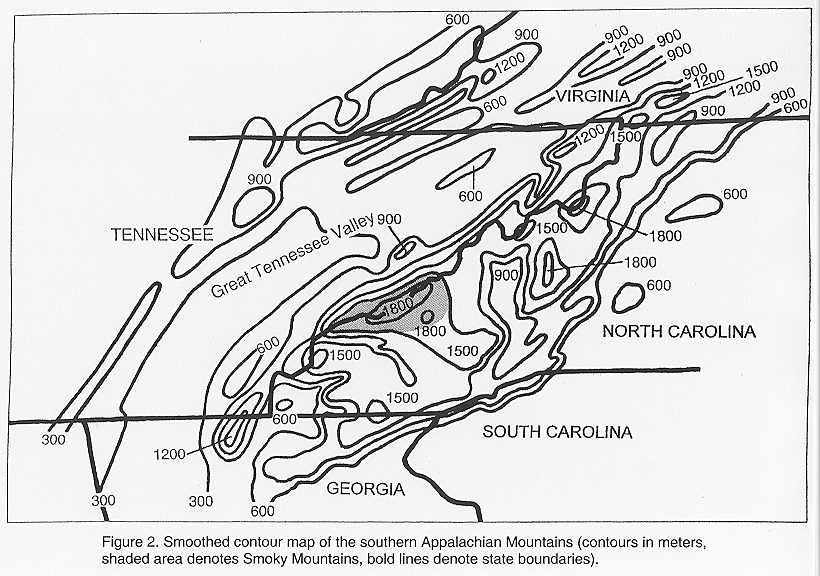
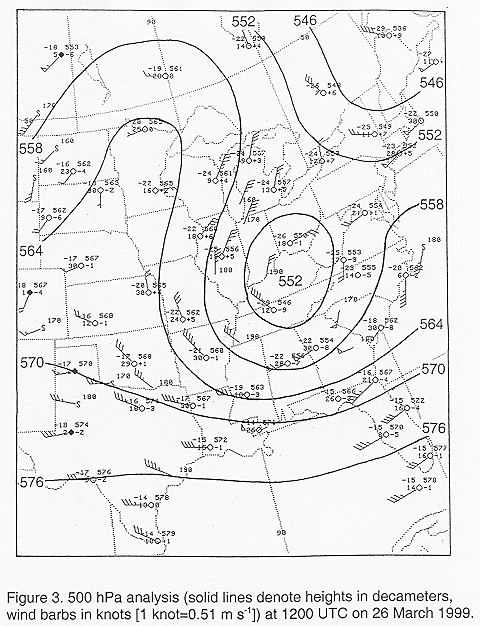
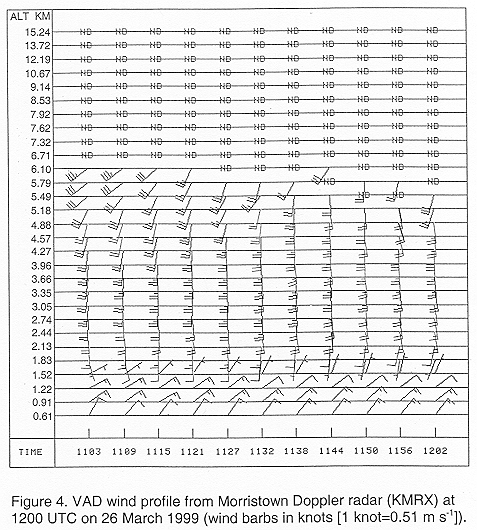
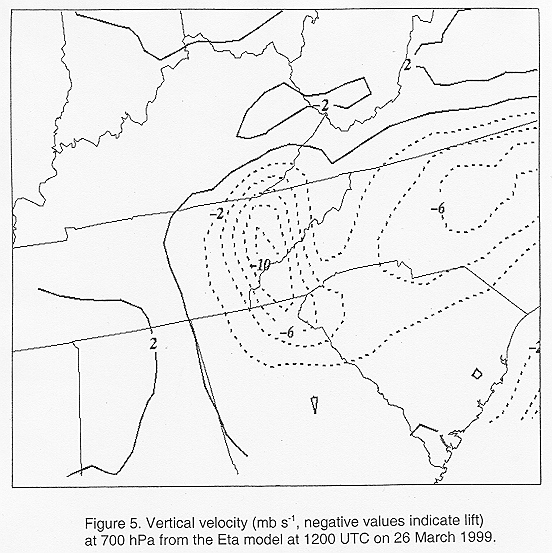
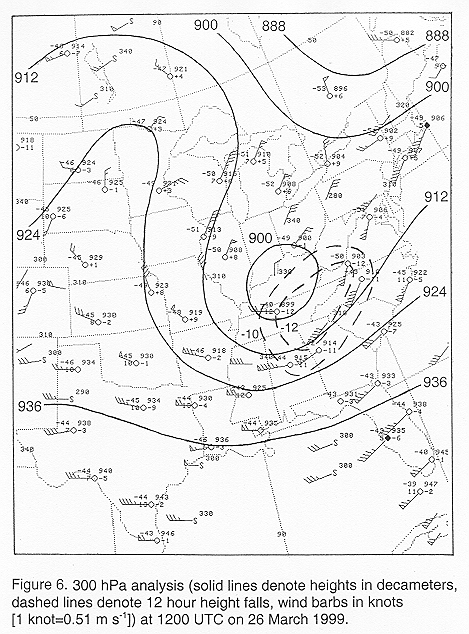
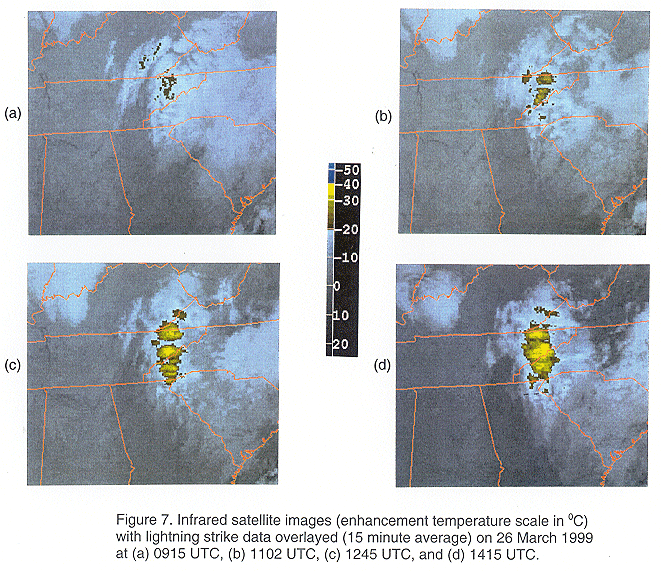
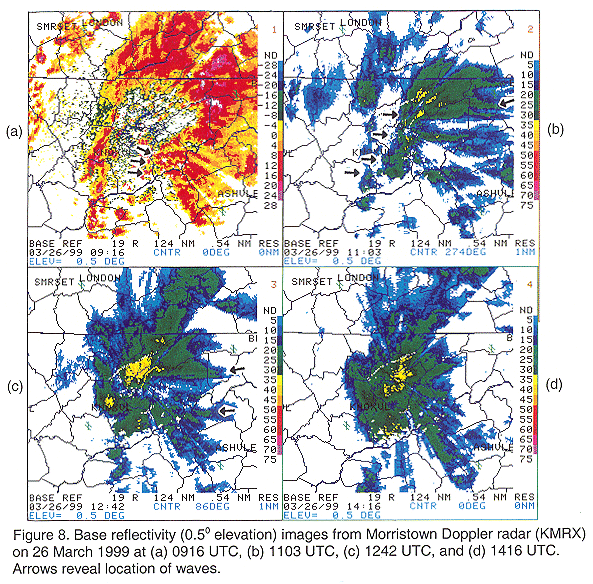
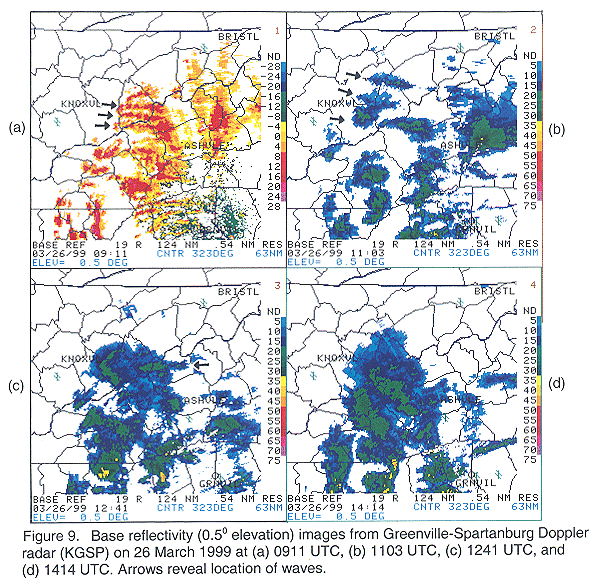
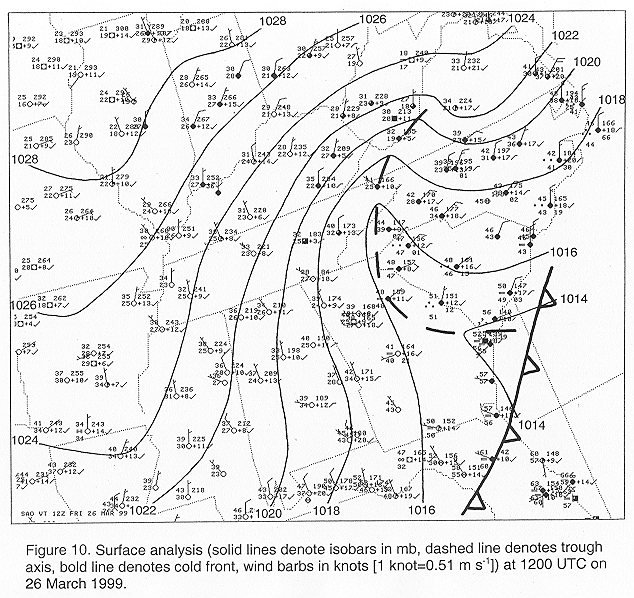
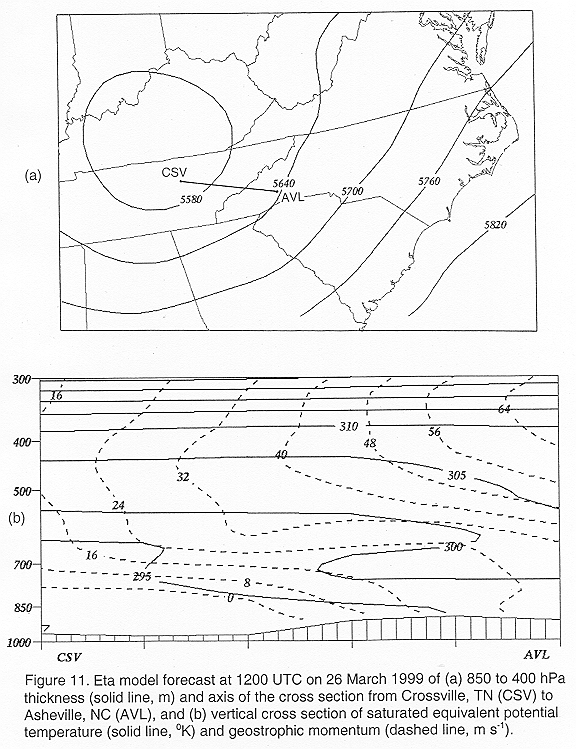
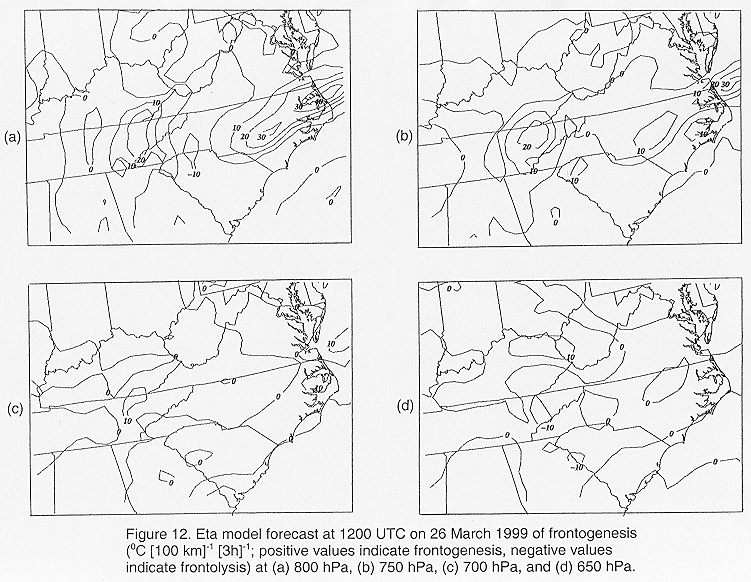
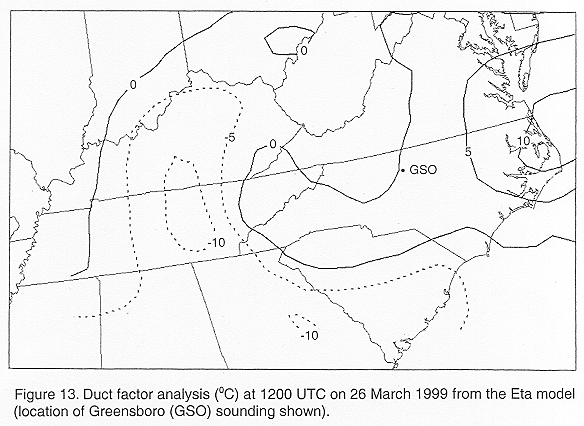
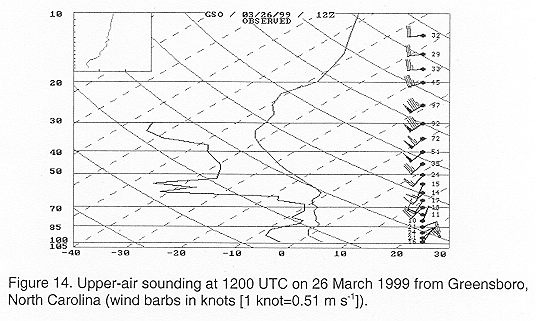
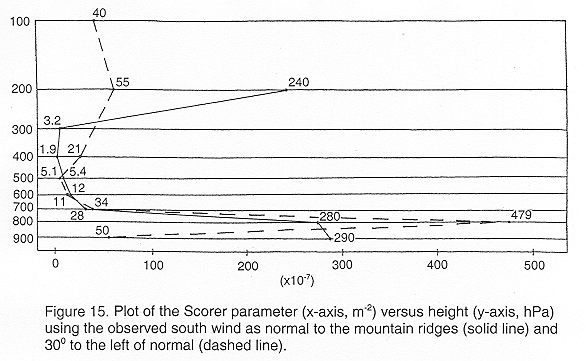
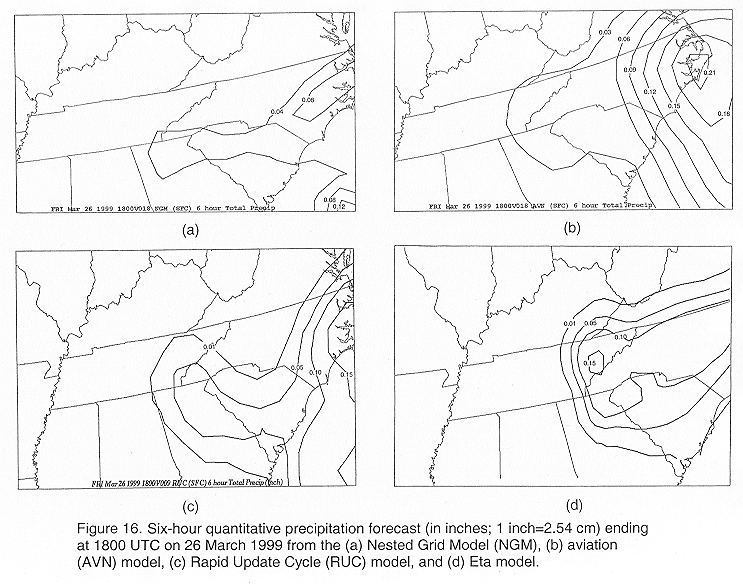